On March 29, 2020, the Los Angeles Times broke the news: 53 members of a community choir in Skagit Valley, Washington—a small town in the northwestern part of the state—had confirmed or suspected COVID-19. A few weeks earlier, the choir’s conductor had encouraged all members to attend rehearsal as long as they showed no signs of illness. The group took precautions: spacing the chairs farther apart than usual, providing hand sanitizer, and avoiding hugs and handshakes. But nobody wore masks.
The Skagit Valley Chorale “exists to enrich the lives of all interested singers and concert audiences . . . expressing the human spirit through choral music.” By practicing for their performance at the upcoming Skagit Valley Tulip Festival, they hoped to spread joy through music. What they spread instead was SARS-CoV-2, the novel coronavirus that causes COVID-19. Within 15 days of their March 10 rehearsal, two choir members had died and 87% of the 61 members present had fallen ill or tested positive.
Taking a collective, measured approach to finding answers calls for building on decades of basic science research—and sometimes looking even further back in history.
What happened in this sleepy Washington community shocked the nation. It was one of the first cases in the U.S. where a group got sick en masse, and scientists could not explain it with the reigning theory of SARS-CoV-2’s spread. Airborne diseases can be transmitted via droplets (large-ish flecks of moisture) or aerosolized particles (which are far smaller); droplets fall to the ground quickly, while aerosols can float in the air for hours. Experts had long accepted that many airborne diseases spread via droplets—that people breathe, sneeze, cough, or sing out flecks of saliva, salt, and virus that splatter on hapless bystanders. This is why doctors wear masks.


Just as John Snow’s famous discovery of a contaminated London well in the 1800s changed our understanding of how cholera spreads (a finding that was initially dismissed by the medical establishment), the Skagit Valley outbreak challenged the conventional wisdom of how viruses like SARS-CoV-2 spread. The droplet-aerosol debate lit up the scientific community during 2020; that’s how science advances, in fits and starts and with lots of discussion. Taking a collective, measured approach to finding answers calls for building on decades of basic science research—and sometimes looking even further back in history.
From pollution to pandemic
When University of Colorado chemist Jose-Luis Jimenez, PhD, saw the LA Times article, he got in touch with the choir right away. The outbreak fit into research he was doing with Virginia Tech environmental engineer Linsey Marr, PhD, and others. “I was working on pollution and climate change and indoor air,” says Jimenez, “and the fact that we had done all that made it possible to contribute to pandemic [research].”
The droplet-aerosol debate is not akin to arguing how many angels can dance on the head of a pin. It has practical meaning for protective measures against the virus, particularly masking, and may explain why the World Health Organization (WHO) and the U.S. Centers for Disease Control and Prevention (CDC) initially told the public not to wear masks.
How could one symptomatic person infect 53 others within a couple of hours in a room the size of a volleyball court, where most people stayed put? He immediately knew the reigning theory could not explain the outbreak.
“WHO was saying that once particles became larger than 5 to 10 microns . . . they behave like projectiles and impact on someone half a meter from you,” or drop to the ground within a meter or two, says Jimenez. “Being familiar with physics, I realized this was extremely erroneous. They don’t need to be 5 microns, they need to be 300 microns to behave like cannonballs, which is a factor of 100,000. At first, I thought it was a typo. And then it was like ‘No, no, no, this is what they think.’”
Jimenez once tweeted that if a virus is the size of a squirrel, WHO says droplets start at the size of an elephant—but really, Jimenez observes, droplet behavior starts at the size of Godzilla. And whether viral particles are, relatively speaking, squirrels, elephants, or Godzillas makes a world of difference in how they behave in the air.
Evidence from the scene
After gathering evidence about the choir rehearsal, Jimenez crunched some numbers; something seemed amiss. How could one symptomatic person infect 53 others within a couple of hours in a room the size of a volleyball court, where most people stayed put? He immediately knew the reigning theory could not explain the outbreak.
“When the light was from a certain direction, [you] could see . . . a continuous spray of saliva coming from the mouth of the surgeon while he . . . conducted his operation.” She became one of the first to recommend that doctors wear masks during surgery.
Rather, Jimenez and Marr saw it as a textbook example of aerosolized dispersion of virus-laden particles. If such particles were smaller than droplets, they’d follow air currents, similar to how cigarette smoke disperses. The cloud of particles would be denser near the smoker (or sick person)—so someone standing nearby would be more likely to breathe in the particles—but the cloud would spread out rapidly following the laws of turbulent diffusion. Coincidentally, these laws also explain the aerosolized dispersal of particulate air pollution or pollen.
In 1905, Chicago physician Alice Hamilton, MD, published a paper describing a medical student’s observation: “When the light was from a certain direction, [you] could see . . . a continuous spray of saliva coming from the mouth of the surgeon while he . . . conducted his operation.” She became one of the first to recommend that doctors wear masks during surgery.
“For every [saliva droplet] you can see,” says Marr, “there are hundreds of much smaller microscopic droplets, and those have been shown to carry virus.” One minute of vigorous speaking—or singing—can generate more than 1,000 virus-containing aerosols that remain airborne for eight minutes or longer.
Marr has spent years studying airborne microbe dispersal—research that grew from her early work on nanoparticles in consumer spray products. “I was able to apply my knowledge of particulate air pollution and what we were studying about engineered nanomaterials to viruses,” she says. “Viruses are really nanoparticles: natural biological nanoparticles of 100 nanometers or less.” But viruses don’t float naked in the air. They’re expelled with body fluids in particles in the range of microns—1,000 times larger than a nanometer. Small droplets under 100 microns, sometimes called droplet nuclei, drift about quite differently from larger, projectile-like ones.
“I think this is where private funders can . . . have a big impact—by funding this research that [falls] between the cracks of the big governmental funding agencies,” Marr says. Both she and Jimenez have received grants from the Alfred P. Sloan Foundation.
Until the pandemic hit, Marr’s research fell between disciplinary silos, which made it hard to find funding for it. It didn’t fall under the National Institutes of Health (NIH), she says, because “they only [study] viruses in the body, and I’m looking at viruses outside the body that behave like particulate air pollution.” It wasn’t the domain of the Environmental Protection Agency, because they avoid airborne (though not waterborne) pathogens. And it’s too health-oriented for the National Science Foundation (NSF). “I think this is where private funders can . . . have a big impact—by funding this research that [falls] between the cracks of the big governmental funding agencies,” Marr says. Both she and Jimenez have received grants from the Alfred P. Sloan Foundation.
In August 2020, Marr joined the NIH’s top infectious disease expert, Anthony Fauci, MD, in a virtual workshop titled “Airborne Transmission of SARS-CoV-2.” Sponsored by the National Academies of Sciences, Engineering, and Medicine, it convened experts from aerosol science, atmospheric chemistry, building engineering, epidemiology, environmental health, infectious diseases, pulmonary medicine, public health, and virology to discuss the role of aerosols in the pandemic—a major move toward greater acceptance of their importance. The workshop was funded by the Gordon and Betty Moore Foundation, the Walmart Foundation, and other sources.
The debate over COVID-19’s spread and the importance of masks—relative to handwashing and social distancing—highlights the wisdom of bringing together people from various disciplines to share their knowledge. Such efforts also sometimes call for a journey back in time.
An error oft-repeated becomes dogma
Jimenez and Marr trace the dismissal of aerosol disease transmission to the early 20th century, though the link between disease and air goes back further still. “The longstanding error comes out of 1910,” says Jimenez, “when a public health researcher, Charles Chapin, found it convenient to say that it is almost impossible for [pathogens] to be transmitted through the air—and that [was] dogma until this pandemic.”
.jpg)
Blaming disease on bad air dated to Hippocrates, who lived from 460 to 377 BCE; he promoted the idea of four humors (yellow bile, black bile, phlegm, and blood), a concept with parallels in traditional Chinese medicine. Whenever plagues struck, people blamed miasmas from rotting carcasses, mold, or dust, which they believed disrupted the humors.
Miasma theory is actually tied to an early use of masks for protection against disease: From the 14th to the 17th centuries, doctors wore beak-shaped masks filled with herbs and spices to ward off supposedly disease-bearing bad odors.
The use of beaked masks fell away after the 17th century, but the public continued associating disease with air until the early 19th century, a grand era of scientific discovery. In the 1860s, French microbiologist Louis Pasteur proposed the germ theory of disease. It was confirmed in the 1880s by German physician Robert Koch, who found that specific microbes cause specific diseases (his work on one such disease, tuberculosis, won him the Nobel Prize). But as misinformation tends to do, the idea of miasma stayed in the public consciousness much longer—hence Chapin’s desire to put it to bed in the early 20th century.
An influential researcher, Chapin authored The Sources and Mode of Infection, reviewing 50 years of evidence since Pasteur and observing that social distancing reduces the risk of infection. He noted two possible explanations for this: microbes were transmitted in either large droplets or small aerosols. He admitted there was no evidence for lack of aerosol disease transmission but nevertheless concluded it was almost impossible, stating, “It will be a great relief to most persons to be freed from the specter of infected air.”
Public masking recommendations have evolved during the course of the pandemic, as more has been learned, but it was always known that medical workers treating COVID-19 patients needed masks.
“I think there was this need to reject miasma theory,” says Marr, “and then there were some influential papers and books where they wanted to emphasize handwashing. There was a lack of understanding that aerosols were present and how they would move. There was some fear associated with that, too: If it’s in the air, that means it could be anywhere and hard to control. So that led to this bias against” the idea of aerosol transmission.

But thinking on the subject has been gradually shifting. In 2003 in Hong Kong, for example, one man with diarrhea spread SARS to 329 others in the same building, 33 of whom died. Some experts suspected aerosolized toilet plumes. COVID-19 super-spreader sites have included cruise ships, religious gatherings, and a homeless shelter. Like the Skagit Valley rehearsal, these cases are difficult to explain by any means but aerosol transmission. No one disagrees that larger airborne particles can and do spread diseases like the common cold, flu, and even COVID-19. But in no small part due to research stimulated by the pandemic, the role of aerosol transmission is now being actively studied.
Corona-charged microfibers
Public masking recommendations have evolved during the course of the pandemic, as more has been learned, but it was always known that medical workers treating COVID-19 patients needed masks: They were in the line of fire from patients’ exhalations, whether from splattering droplets or aerosol clouds.
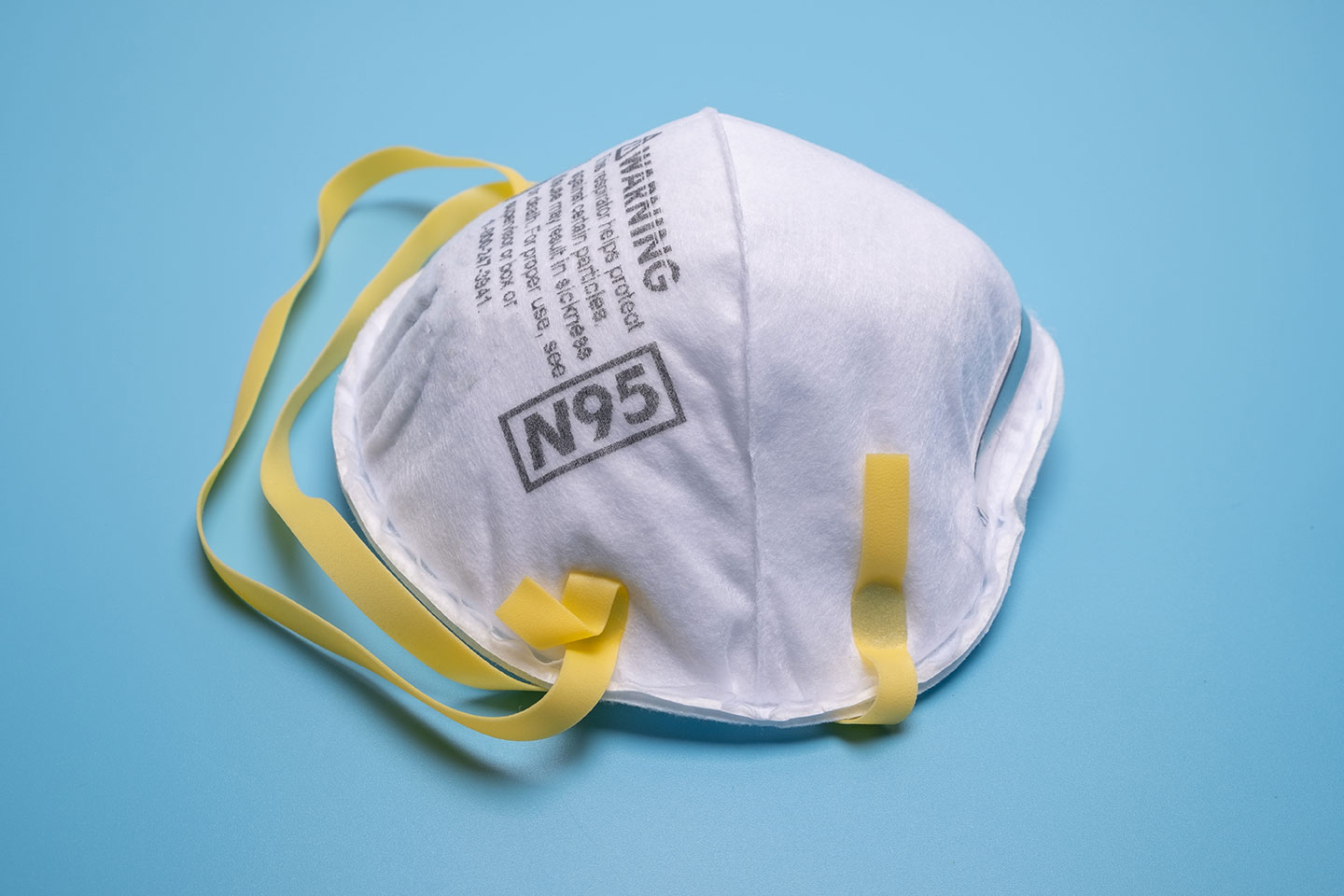
Yet given the sudden increase in demand, the mask supply chain couldn’t keep up. Properly worn surgical masks are fairly effective at keeping out (or in) aerosols, filtering about 80% of aerosolized particles. But tight-fitting, electrically charged N95 masks have long been the gold standard for medical workers.
The N95 wouldn’t exist were it not for retired University of Tennessee materials scientist Peter Tsai, PhD. In the 1990s, Tsai patented a process to electrically charge micro-woven fabric, coincidentally called a corona charge. “Tangled mats of microfibers made of polypropylene are good materials for air filters—such as those in N95 respirators,” he said in a Nature profile. “I invented a way to charge those filters by passing them through a device that produces static electricity—much like when you rub a balloon on your hair, but it’s permanent.” The charge makes the material 10 times more efficient at blocking particles.
The first masks made with Tsai’s charged polypropylene were for protection from particulate air pollution, but the fabric was soon used to protect medical workers, too. N95 masks capture at least 95% of airborne particles, but they’re meant for one-time use. When the pandemic struck, Tsai emerged from retirement to help scientists develop methods to sterilize N95 masks. He found that heating the masks at 70°C for an hour, boiling them for five minutes, or using a mask only every three or four days can get medical workers through a pinch.
Why are so many masks made of polypropylene? “Polypropylene is dirt cheap. It can be made in ridiculous quantities. It’s really good at fiber formation. It’s easy to melt. It’s got all the physical properties you would want,” says Edmund Palermo, PhD.
The key to N95 masks’ success lies in the material: meltblown polypropylene. “You essentially melt a large vat of polypropylene, and you spit it out at really high rates at a surface, such that you get these really fine fibers randomly arranged into a mask,” explains Helen Zha, PhD, a materials scientist at Rensselaer Polytechnic Institute. They’re like minuscule strands of spaghetti; the complex structure traps particles, while leaving space for breathability.
More recently, Stanford materials scientist Yi Cui, PhD, and others have developed masks made of polymer nanofibers, which are one-twentieth to one one-hundredth the size of microfibers. “You have high [filtration] efficiency, but your porosity is maintained at 95% or higher, so your air breathability is very good,” he says. Together with Physics Nobel Laureate Steven Chu, PhD, Cui cofounded 4C Air and invented a nanofiber-based mask with “the world record for the best breathability,” using a process called electrospinning.
A spontaneous invention leads to a Nobel Prize
Why are so many masks made of polypropylene? “Polypropylene is dirt cheap. It can be made in ridiculous quantities. It’s really good at fiber formation. It’s easy to melt. It’s got all the physical properties you would want,” says Edmund Palermo, PhD, who like Zha is a materials scientist at Rensselaer. Polypropylene emerged in the 1950s thanks to work by chemists Karl Ziegler, PhD, Giulio Natta, PhD, and others—who discovered, says Palermo, “the kind of catalysts that can polymerize propylene into a useful form.”
“It’s sometimes tricky to get funding to do this kind of basic science research because it doesn’t sound sexy. If I say I want to do some research on how polymers interact with surfaces, it sounds less sexy than saying I want to make thin-film coatings that kill viruses on filters, but they go hand in hand.”
Ziegler (who shared the Nobel Prize for Chemistry with Natta in 1963 for their work with polymers) had no idea his research would lead to something so practical. In his Nobel lecture, Ziegler said, “I have never started with anything like a formally presented problem. The whole effort developed quite spontaneously. It was pure chance that in November of 1953 the first of the catalysts in which our invention was clearly recognizable happened to be a relatively weak-acting combination of an aluminum alkyl with a zirconium compound, by which ethylene could be polymerized only under a few atmospheres pressure.” Before his experiments, creating polyethylene required ultra-high pressures of 1000 to 2000 atmospheres, and the resulting plastic was rigid.
“I’m sure that when Ziegler and Natta were developing polymerization catalysts in the 1940s,” says Palermo, “they were not thinking of N95 masks.” Nevertheless, flexible polypropylene was key to creating meltblown fabrics and, later, nanofibers.
“You never know what direction [research] is going to go in,” he says, “and how it’s going to have an impact four decades later when a pandemic rolls around.”
“Maybe a question that we should wonder is, why were no antimicrobial polymers ever used in the development of these kinds of masks,” posits Zha, whose project with Palermo to add permanently charged antimicrobial polymer coatings to N95 filters was funded by the NSF. “It’s sometimes tricky to get funding to do this kind of basic science research because it doesn’t sound sexy. If I say I want to do some research on how polymers interact with surfaces, it sounds less sexy than saying I want to make thin-film coatings that kill viruses on filters, but they go hand in hand.” Her prior research on molecular self-assembly, including spider silk, gave her the know-how to attach these novel coatings to mask surfaces.
“What we’re trying to do is make synthetic polymers that mimic host-defense peptides that are present in the innate immune system,” says Palermo. “When a bacterial infection is detected, the body releases these peptides—short-chain polymers of amino acids that directly kill the bacteria.”
The study of host-defense peptides was spurred by a serendipitous finding. In 1987, translational scientist Michael Zasloff, MD, PhD, discovered such peptides in African clawed frogs. “He’d do surgery on frogs and then put them back in this microbially contaminated pond water, and he wondered, ‘I cut the frog open, and I put it back in dirty water, and it never gets an infection,’” explains Palermo. Zasloff then isolated a peptide, a potent antibacterial agent, from the frog’s skin. Isolation of similar host-defense peptides led chemists like Palermo to start researching synthetic polymers that mimic natural ones. “You never know what direction [research] is going to go in,” he says, “and how it’s going to have an impact four decades later when a pandemic rolls around.”
Zha and Palermo’s research could lead to better, reusable masks, offering both environmental and health benefits. N95 masks were designed to be disposable. “Not only is that putting a large pressure on the supply chain in terms of a pandemic like this, it’s incredibly bad for the environment,” says Zha. “Polypropylene is a nonbiodegradable plastic. And I think moving towards the future that we all like to live in, even aside from scarcity and shortages during pandemic times, we as a society should still be invested in developing filtration materials that enable them to be used over and over.”
Could masks have prevented the Skagit Valley outbreak?
There’s no doubt that wearing masks (whether antimicrobial, N95, or surgical) can reduce transmission in both directions—as long as people wear them correctly (over their mouth and nose) and don’t fiddle with them.
Once Jimenez gathered all the details of the Skagit Valley super-spreader event, he and his colleagues developed a mathematical model to describe the airborne spread of SARS-CoV-2—“basically the same thing we use for contaminants, whether carbon monoxide, radon, or mold,” he says. “It’s not rocket science, but it’s confusing to develop it from scratch.” Their easy-to-use COVID-19 Airborne Transmission Estimator has become popular among governments, businesses, and individuals to predict how the size of a space, number of people, ventilation, and mask-wearing affect the likelihood of contracting COVID-19 if someone in the space is infected. It does not account for the increased risk of standing near that person, however.
Today’s scientists stand on the backs of giants. “You can’t solve a problem with just one year,” says Zha. “You always build on decades and decades of previous basic science research.”
Applying his estimator, Jimenez found that if the choir members had worn masks, their risk of infection would have dropped from 87% to 44%. If they’d shortened the rehearsal time and had better ventilation, only 2 people instead of 53 would likely have been infected.
“Masks reduce all modes of transmission,” says Jimenez. “They prevent most droplets and many aerosols from coming out of an infected person. They also block droplets from [hitting] the nose or mouth of a healthy person and reduce the fraction of the aerosols inhaled.”
Nearly a year after Jimenez first heard of the Skagit Valley Chorale, scads of research has pushed scientific progress forward more rapidly than the likes of Chapin and Ziegler could have imagined. In just over a year, scientific thought on the spread of COVID-19 has evolved dramatically. In addition, the SARS-CoV-2 genome has been sequenced, vaccines have been developed, novel antimicrobial and nanofiber masks have been invented, and thousands of scientific studies have been published—but all that didn’t happen by chance. Today’s scientists stand on the backs of giants. “You can’t solve a problem with just one year,” says Zha. “You always build on decades and decades of previous basic science research.”